Spatial learning can become impaired without evidence for neuron loss, whereas eye-blink conditioning deficits are well correlated with Purkinje neuron loss. Glial activation, in particular the increased expression of GFAP, may be a factor in impaired synaptic plasticity. Lastly, I discuss how developmental variations in the numbers of Purkinje cells and ovarian oocytes can be factors in outcomes of aging that are not under strict genetic control.
Cognitive functions show many alternative outcomes during aging. Individual trajectories range from the devastating outcomes of Alzheimer disease (AD) and multi-infarct dementia (MID) to very mild changes detected during middle-age as well as at advanced ages that may not interfere with most activities of daily living. Early indications of age changes in brain functions are well documented during middle-age in clinically healthy individuals, that is in humans aged 35-65 yrs and rodents aged 12-24 mos. These ages precede the main phase of mortality accelerations which is a characteristic of senescence in population [11,22]. For example, psychologists have long recognized that the speed of processing slows relatively early in aging in of adult humans and mammalian models, as discussed at length in James Birren's classic monograph, Psychology of Aging in 1964 [4] and which anticipated a vigorous research area of general importance to cognition in aging [17, 38]. Complex learning paradigms also show marked changes during middle age, e.g. in Paul Bakes' novel paradigm of "Memorizing while Walking"[23].
2. Neuron numbers and functional brain aging
The cellular basis for most cognitive aging changes is not clear, but could in some learning paradigms be independent of neuron loss. Neurons have long been the main focus of study in brain aging because of the clear importance of neuron loss to cognitive deficits in AD and MID. However, certain brain aging changes do not involve neuron loss, as will be discussed below, and begin soon after maturation in the absence of neurological disorders.
Mark West and colleagues have done a series of exacting studies of normal aging. Two year old rats, which are equivalent to the human age group of 65, showed marked individual differences in the extent of cognitive impairments in a maze learning test The brains were examined in a subgroup which was learning-impaired and a subgroup with learning which was indistinguishable from young adults [35]. Examination of hippocampal neuron circuits by stereological techniques for precision counting did not detect any neuron loss. The absence of neuron loss in the zone of CA1 pyramidal neurons is particularly striking, because CA1 neurons are which heavily damaged in AD. Normal humans showed similar stability of CA1 neuron number [49]. Earlier reports of extensive neuron loss during aging in the rodent hippocampus may be due to stress exposure, in view of the correlations between developmental stressors, the sensitivity of the adult hypothalamic-pituitary-adrenal axis, and neuron loss during aging [10, 26, 42].
On the other hand, impairments in eye-blink conditioning in normal aging may involve Purkinje neuron loss in the cerebellum, in studies by Diana Woodruff - Pak. Healthy human adults shows extensive differences in eye-blink conditioning, a reflex pathway which is mediated by the Purkinje cells of the cerebellum. A subgroup emerges during middle-age with slower learning [52]. At later ages, a subgroup of elderly with very slow eye-blink conditioning subsequently became demented (probably AD) [50, 53]. Rabbits, which do not develop Alzheimer-like changes during aging, also show slowed eye-blink conditioned learning during middle age [53]. In this case, there is a strong correlation between the numbers of Purkinje neurons and the efficacy of eye-blink conditioning during aging, but also in young adults. There is generally consistent evidence of Purkinje neuron loss during "normal aging", which in humans is not apparent until after 60 [16, 53], but may begin in early middle-age in rodents by 12 months [36]. The difference between the cerebellum with its more pronounced neuron loss versus the hippocampus with sporadic neuron loss is consistent with the differential vulnerability of neurons to ischemic injury and to AD.
Another important observation is that young adults differ individually by up to 50% in the numbers of Purkinje neurons, as shown directly by neuron counting in rabbits [51]and by MRI estimates of cerebellar volume in humans [54] which both correlate with eye-blink conditioning. Moreover, hippocampal neurons also show a 50% range of variations in rats [26, 35]. The origins of the variations and their possible significance to outcomes of aging is discussed further below.
3. Glial activation during aging
dial changes of normal brain aging have been largely neglected, because of the general assumption that glial activation is secondary to neuron degeneration. However, both astrocytes and microglia become activated early in aging without concurrent diagnosable pathology. My lab is studying the activation of astrocytes during normal aging, using glial fibrillary acidic protein (GFAP) as a marker. GFAP expression increases progressively during aging in humans and inbred lab rodents, as measured by GFAP mRNA and protein [10, 15, 29,30, 32]. Although the cell volume of astrocytes compartment increases during aging, the numbers of astrocytes shows much more modest changes that are not detected in most studies of aging male rodents [10]. Rabbits also showed increased GFAP during aging [53]. The similar increases of GFAP in aging rodents and humans is important, because inbred rodents do not develop Alzheimer changes of solid brain amyloid deposits and hippocampal neurodegeneration unless transfected with human AD genes.
The increased GFAP expression during aging is due to increased transcription of GFAP, as shown by in situ hybridization at a cellular level with intronic cRNA probes [29, 30]. We have developed in vitro approaches to astro -cyte gene expression changes during aging. Primary glia cultures from aging adult cerebral cortex show the persistence of in vivo activation, e.g. astrocytes from 12-mo vs 3 mo old rat cortex show 2-fold higher GFAP transcription when transfected with a full length GFAP promoter [10]. We hypothesize that GFAP transcription increases during aging are caused by the increased load of oxidatively damaged proteins, which occurs in tissues throughout the body during aging, as well as in the brain [9, 10, 29, 41]. The level of oxidative damage during aging is decreased by reducing average caloric intake through "caloric restriction" paradigms which robustly slow many aging processes inversely to the caloric restriction over a 10-40% range in laboratory rodents [10, 41].
Caloric restriction also attenuates glial activation during aging, including GFAP transcription, which we showed by in situ hybridization using GFAP intron cRNA [29, 30]. Activation of the GFAP promoter by oxidative stress (hydrogen peroxide, cysteamine) [29] may be mediated by a functional NFkB-NF1 site in the upstream GFAP promoter [Wei, Morgan, Finch, in prep.], which is known to mediate responses to oxidative stress in other genes. The GFAP promoter has highly conserved domains in humans and rodents [21] which could mediate certain shared (canonical) features of brain cell aging changes shared in short- and long-lived mammals. The ensemble of oxidative and inflammatory processes of aging throughout the body can be considered as a "gero-inflammatory manifold", which may underlie AD and many other age-related diseases [9, 10].
GFAP expression is usually regarded as a secondary to neurodegeneration. However, we are evaluating the possibility that the increased expression of GFAP during aging may contribute to decreased synaptic functions. An important clue comes from the wounding-in-a-dish model, in which fetal neurons are cocultured with astrocytes [22, 25,37]. A scratch wound causes immediate astrocyte reactivity. Neurite outgrowth is greatly enhanced by treatment with antisense GFAP which decreases astrocyte fibrosis and also reorganization of astrocytic extracellular laminin [6, 21, 37]. Similarly, neurite outgrowth was enhanced in astrocytes from the GFAP-KO genotype, which have lower GFAP expression [27].
4. Sex steroids and sprouting
We are using the wounding-in-a dish model analyze sex-steroid sensitive aspects of neuronal sprouting and effects of aging. in vivo, we showed that the presence of estradiol increases sprouting in the hippocampus after perforant path lesions which are a model for hippocampal deafferentation during AD [43, 44]. The sprouting responses depend on the presence of apolipoprotein E (apoE), as shown by apoE knockout mice [43, 47]. Further, we are developing the hypothesis that sprouting also is enhanced by estradiol through its inhibition of GFAP induction after the lesion [37, 44]. In the wounding in a dish model, we showed that GFAP transcription and protein are repressed by estradiol, in association with increased sprouting and laminin reorganization [37], just as obtained by antisense treatment [6, 22]. The effects of E2 on GFAP transcription depend on a functional estrogen response element in the upstream promoter [37] and interactions with other loci in highly conserved regions of the GFAP promoter [21]. These findings support the hypothesis that the enhancement of neuronal sprouting by E2 is driven by the repression of GFAP transcription, which in turn decreases intermediate filament formation with further downstream effects on laminin reorganization, i.e. the inverse relationship in astrocytes between GFAP expression and pro-neuritogenic laminin expression.
From the two paradigms of GFAP decrease (E2-repression, antisense) which have the same effects on laminin reorganization and neurite outgrowth, we propose that GFAP expression is inverse to the pro-neuritogenic organization of laminin in astrocytes. This hypothesis also predicts an interaction with aging, because GFAP expression increases per astrocyte during aging. The inverse GFAP-laminin hypothesis predicts that the laminin reorganization in wounding responses should be diminished in astrocytes derived from aging brains.
Responses to perforant path lesions showed complex effects of aging in females: the induction of neuronal sprouting was impaired in aging females and, unlike young adults, was not sensitive to estradiol of ovariectomy. In astrocytes, GFAP induction was greater than in young, whereas apoE was diminished, which both could contribute to the impaired sprouting [34]. In view of the inverse association of astrocytic GFAP expression with neuronal sprouting in the above culture model and in many in vivo situations, we hypothesize that the increased expression of GFAP in aging females is a factor in the impaired sprouting. Preliminary results in the wounding-in-a-dish model show that astrocytes from aging mice are not responsive to estradiol in supporting neuron outgrowth, as observed in vivo.
I suggest further that there may be general relationships between astrocyte hyperactivity during aging and some neuronal functions which are modified by increased astrocyte contact with neurons. In brief, several lines of evidence indicate that astrocyte coverage of neurons can modifies synaptic activity by controlling local neurotransmitter concentrations [1, 33, 37]. It is possible that these mechanisms contribute to the effects of 'glial scarring' from the astrocyte hypertrophy that has long-been implicated in inhibiting neurite outgrowth in adult brain injury.
We may then consider possible interactions between glial activation and the concurrent changes observed to begin soon after maturation in mild regressions of neuro -transmitter binding sites in all mammals examined. One branch of this large literature concerns various monoaminergic receptor binding sites. We showed that decreases in certain monoamine binding sites begin soon after maturation in cortical and subcortical regions of normal humans and rodents, e.g. D2 binding dopaminergic sites [20, 28, 40] The D2 site loss with normal aging was shown by PET to parallel the postmortem findings across the adult life span in several studies of normal humans [19, 46, 48]. PET studies of D2 sites in the rat also showed deficits in middle-age [19]. The functional significance of these age decreases in D2 binding sites is indicated by age changes in drug sensitivity. For example, quinpirol antagonism of cortical D2 sites which mediate delayed recall becomes progressively more sensitive in aging monkeys 10, 20, and 40 years [2]. Moreover, glucose metabolism shows correlations with the density of D2 sites measured by PET in normal humans, which, again, shows a subgroup with lower values emerging during middle-age [48]. We may anticipate that age changes in monoamine systems that influence multi-tasking and other executive functions will become a major topic of research and pharmacologic intervention. I suggest the outline of a general hypothesis of brain aging, in which slowly accumulating oxidative damage activates astrocytic transcription of GFAP and other glial genes. The increased GFAP expression increases astrocyte volume, which in turn expands the are of neuron surfaces that are contacted by astrocyte membranes. Although it is premature to discuss the many possible cellular consequences of increased astrocyte-neuron contact, the experimental evidence clearly shows effects of astrocyte coverage of neurons on neurotransmitter functions [1, 33, 37].
5. Inidividual outcomes in aging and developmental variations in cell numbers
Lastly, I briefly discuss the issue of heritability in outcomes of brain aging, which can be understood, I suggest, in terms of chance developmental variations that influence brain cell numbers. This material draws from my recent monograph co-authored with Tom Kirkwood: Chance, Development, and Aging [8]. An important example of nonheritable individual variations in brain aging is given by identical (MZ) twins who show major differences in the onset age of clinical dementia. In Particular, Swedish MZ twins concordant for dementia differed by a mean of 4.5 years; one concordant twin pair differed in dementia onset by 16 years [14]. Moreover, differences between MZ twins in outcomes of cognitive aging are described for nondementing cognitive changes: ".....idiosyncratic experience [was] the environmental component that most determines individual differences in cognitive abilities late in life" [24]. I suggest that differences in neuron numbers is another source of individual variations within MZ twin pairs which would otherwise be attributed to 'idiosyncratic experience'. Many cell populations differ in numbers within identical genotypes due to developmental variations [9]. Data are strongest for neurons and for ovarian oocytes which define at birth the reserves against which individuals draw.
In young healthy adult rabbits, the number of Purkinje neurons is implicated as the basis for intersubject variability in learning curve for eye-blink conditioning, with strong correlations of conditioning efficacy with individual differences in Purkinje cell density that arose during development [51]. Young humans show similar correlations of cerebellar volume with eye-blink conditioning [54], which implicates variations in Purkinje in cell number. The Purkinje system is the first clear example of developmental -ly-originating differences in neuron number in a specific circuit in neurologically normal adults that determine quantitative variation of a brain function. As another example, MZ twin pairs show substantial variation in the hippocampal volume [9, 34] Variations in hippocampal neuron reserve between individuals could be the basis for the co-MZ twin differences in the onset of clinical impairments in memory.
Female reproductive aging also shows major differences between genetically identical individuals which can be attributed to chance developmental variations in the numbers of oocytes and follicles as determined before birth. For example, inbred mice of several strains show a >2-fold variability between individuals in the numbers of oocytes and primary follicles in the ovary at birth, which we hypothesize is the major factor in individual variations of the age of oocyte depletion during middle-age [9]. The argument is based on an elegant experiment by my former students James Nelson and Leda Felicio [31] that tested the relationship between the numbers of oocytes present in the young ovary to the time of later life reproductive senescence. This study diminished the reserve oocyte pool in young adults by 'radical ovarian resection' or surgical removal of most of the ovary. The rate of loss of ovarian oocytes during postnatal aging approximates zero-order kinetics, like radioactive decay, and can be fitted nicely to the linear regression of log (oocytes) vs. age, from which it can be calculated that a 90% reduction of oocyte loss by surgical resection should cause the acceleration of reproductive senescence by about 7 months. The results from longitudinal daily observations of vaginal cytology confirmed this prediction for both the age-related lengthening of cycles as well as the onset of acyclicity. Thus, variations in the numbers of oocytes that arise before birth in humans are a key factor in the age of natural menopause five decades later. In turn, individual variations in the age at menopause are risk factors of morbidity and mortality, particularly osteoporosis with subsequent increased risks of disabling fractures of bone and changes in blood lipids that accelerate atherogenesis with increased risks of heart attacks and strokes. In these conditions, estrogen replacement therapy (ERT) shows generally consistent risk reduction. A recent addition to the list of menopause-associated causes of mortality is the possibility that the risk of AD in women can be reduced by ERT [18, 39]. An open question is how the age at menopause may interact with the onset of AD. In view of the major effects of blood estradiol levels on hippocampal synaptic efficacy and long-term potentiation [3, 12], detailed investigations are needed of relationships between the duration of estrogen deficiencies after menopause and cognitive changes. These questions may extend to males, in view of associations of cognitive performance and plasma estradiol [5] and the efficacy of estradiol on long-term potentiation in young adult male rat hippocampal slices [12].
Acknowledgements
This work was supported by grants to the author from the National Institute on Aging (AG 09793, AG-13499; AG-14571), the Alzheimer's Association, and the John Douglas French Foundation for Alzheimer disease.
References
Araque A., Carmignoto G., Haydon P.G.: Dynamic signaling between astrocytes and neurons. Ann. Rev. Physiol. 2001;63:795-813.
Arnsten A.F., Cai J.X., Steere J.C., Goldman-Rakic P.S.:
Dopamine D2 receptor mechanisms contribute to age-related cognitive decline: the effects of quinpirole on memory and motor performance in monkeys. J Neurosci 1995; 15:3429-3439.Bi R., Foy M.R., Vouimba R.M. et al.: Cyclic changes in estradiol regulate synaptic plasticity through the MAP kinase pathway. Proc Natl Acad Sci U S A 2001;98:13391-13395.
Birren J.E. Englewood Cliffs, NJ, Prentice-Hall, 1964.
Carlson L.E., Sherwin B.B.: Higher levels of plasma estradiol and testosterone in healthy elderly men compared with age-matched women may protect aspects of explicit memory. Menopause 2000;7:168-177.
Costa S., Planchenault T., Charriere-Bertrand C. et al.:
Astroglial permissivityfor neuritic outgrowth in neuron-astrocyte cocultures depends on regulation of laminin bioavailability. Glia 2002;37:105-113.Finch C.: Longevity, Senescence, and the Genome, U Chicago Press, 1990.
Finch C., Kirkwood T.B.L: Chance, Development, and Aging, Oxford University Press, 2000.
Finch C., Longo V.: The Gero-inflammatory manifold; in Roger J (ed): Neuroinflammatory Mechanisms in Alzheimer's Disease: Basic and Clinical Research, Basel: BirkhauserVerlag,
2000, pp Chapter 1.Finch C., Morgan T.E., Rozovskyl., Xie Z. et al.: Microglia and aging in the brain.; in Streit WJ (ed): Microglia in the Regenerating and Degenerating Central Nervous System. New York, Springer-Verlag, 2002, pp 275-305.
Finch C.E., Pike M.C., Witten M.: Slow mortality rate accelerations during aging in some animals approximate that of humans. Science 1990;249:902-905.
Foy M.R., Xu J., Xie X. et al.: 17beta-estradiol enhances NMDA receptor-mediated EPSPs and long-term potentiation. J Neurophysiol 1999;81:925-929.
Garcia Segura L.M., Naftolin F., Hutchison J.B., et al.:
Role of astroglia in estrogen regulation of synaptic plasticity and brain repair. J Neurobiol 1999;40:574-584.Gatz M., Pedersen N.L., Berg S. et al.: Heritability for Alzheimer's disease: the study of dementia in Swedish twins. J Gerontol A Biol Sci Med Sci 1997;52:M117-125.
GossJ.R., Finch C.E., Morgan D.G.: Age-related changes in glial fibrillary acidic protein mRNA in the mouse brain. Neurobiol Aging 1991;12:165-170.
Hall T.C., Miller A.K.H., Corsellis J.A.N.: Variations in the human Purkinje cell population according to age and sex. Neuropathol Appl Neurobiol 1975;1:267-292.
Hof P.R., Mobbs C.V.: Functional Neurobiology of Aging.
2001.Hogervorst E., Williams J., Budge M., RiedelW., JollesJ.:
The nature of the effect of female gonadal hormone replacement therapy on cognitive function in post-menopausal women: a meta-analysis. Neuroscience 2000;101:485-512.Inoue M., Suhara T., Sudo Y., Okubo Y. et al.: Age-related reduction of extrastriatal dopamine D2 receptor measured by PET. Life Sci 2001;69:1079-1084.
Keck B.J., Lakowski J.M.: Neurochemistry of receptor dynamics in the aging brain: in Hof PR, Mobbs CV (eds):
Functional Neurobiology of Aging. New York, Academic Press, 2001, pp 21-29.Laping N.J., Teter B., Nichols N.R., Rozovsky I., Finch C.E.: Glial fibrillary acidic protein: regulation by hormones, cytokines, and growth factors. Brain Pathol 1994;4:259-275.
Lefrancois T., Fages C., Peschanski M., Tardy M.:
Neuritic outgrowth associated with astroglial phenotypic changes induced by antisense glial fibrillary acidic protein (GFAP) mRNA in injured neuron-astrocyte cocultures. J Neurosci 1997;17:4121-4128.Lindenberger U., Marsiske M., Baltes P.B.: Memorizing while walking: increase in dual-task costs from young adulthood to old age. Psychol Aging 2000;15:417-436.
McClearn G.E., Johansson В., Berg S. et al.: Substantial genetic influence on cognitive abilities in twins 80 or more years old. Science 1997;276:1560-1563.
McMillian M.K., Thai L, Hong J.S. et al.: Brain injury in a dish: a model for reactive gliosis. Trends Neurosci 1994;17:138-142.
Meaney M.J., Aitken D.H., van Berkel C., Bhatnagar S., Sapolsky R.M.: Effect of neonatal handling on age-related impairments associated with the hippocampus. Science 1988;239:766-768.
Menet V., Gimenez у Ribotta M., Chauvet N. et al.:
Inactivation of the glial fibrillary acidic protein gene, but not that of vimentin, improves neuronal survival and neurite growth by modifying adhesion molecule expression. J Neurosci 2001;21:6147-6158.Morgan D.G., Marcusson J.O., Nyberg P. et al.: Divergent changes in D-1 and D-2 dopamine binding sites in human brain during aging. Neurobiol Aging 1987;8:195-201.
Morgan Т.Е., Rozovsky I., Goldsmith S.K., et al.:
Increased transcription of the astrocyte gene GFAP during middle-age is attenuated by food restriction: implications for the role of oxidative stress. Free Radic Biol Med 1997;23:524-528.Morgan Т.Е., Xie Z., Goldsmith S., Yoshida Т., et al.: The mosaic of brain glial hyperactivity during normal ageing and its attenuation by food restriction. Neuroscience 1999;89:687-699.
Nelson J.F., Felicio L.S.: Radical ovarian resection advances the onset of persistent vaginal cornification but only transiently disrupts hypothalamic-pituitary regulation of cyclicity in C57BL/6J mice. Biol Reprod 1986;35:957-964.
Nichols N.R., Day J.R., Laping N.J., Johnson S.A., Finch C.E.: GFAP mRNA increases with age in rat and human brain. Neurobiol Aging 1993;14:421-429.
Oliet S.H., Piet R., Poulain D.A.: Control of glutamate clearance and synaptic efficacy by glial coverage of neurons. Science 2001;292:923-926.
Plassman B.L, Welsh-Bohmer K.A., Bigler E.D. et al.:
Apolipoprotein E epsilon 4 allele and hippocampal volume in twins with normal cognition. Neurology 1997;48:985-989.Rasmussen Т., Schliemann Т., Sorensen J.C. et al.:
Memory impaired aged rats: no loss of principal hippocampal and subicular neurons. Neurobiol Aging 1996;17:143-147.Rogers J., Zornetzer S.F., Bloom F.E., Mervis R.E.:
Senescent microstructural changes in rat cerebellum. Brain Res 1984;292:23-32.Rozovsky I., Wei M., Stone D.J., Zanjani H. et al.: Estradiol (E2) enhances neurite outgrowth by repressing glial fibrillary acidic protein expression and reorganizing laminin. Endocrinology 2002;143:636-646.
Salthouse Т.A.: Aging and measures of processing speed. Biol Psychol 2000;54:35-54.
Schneider L.S., Finch C.E.: Can estrogens prevent neurodegeneration? Drugs Aging 1997;11:87-95.
Severson J.A., Finch C.E.: Reduced dopaminergic binding during aging in the rodent striatum. Brain Res 1980;192:147-162.
Sohal R.S., Weindruch R.: Oxidative stress, caloric restriction, and aging. Science 1996;273:59-63.
Stein-Behrens В., Mattson M.P., Chang I., Yeh M., Sapolsky R.: Stress exacerbates neuron loss and cytoskeletal pathology in the hippocampus. J Neurosci 1994;14:5373-5380.
Stone D.J., Rozovsky I., Morgan Т.Е. et al.: Increased synaptic sprouting in response to estrogen via an apolipoprotein E-dependent mechanism: implications for Alzheimer's disease. J Neurosci 1998;18:3180-3185.
Stone D.J., Rozovsky I., Morgan Т.Е., Anderson C.P. et al.: Effects of age on gene expression during estrogen-induced synaptic sprouting in the female rat. Exp Neurol 2000;165:46-57.
Stone D.J., Song Y., Anderson C.P., Krohn K.K., Finch C.E., Rozovsky I.: Bidirectional transcription regulation of glial fibrillary acidic protein by estradiol in vivo and in vitro. Endocrinology 1998; 139:3202-3209.
Suzuki M., Hatano К., Sakiyama Y. et al.: Age-related changes of dopamine D1-like and D2-like receptor binding in the F344/N rat striatum revealed by positron emission tomography and in vitro receptor autoradiography. Synapse 2001;41:285-293.
Teter В., Harris-White M.E., Frautschy S.A., Cole G.M.:
Role of apolipoprotein E and estrogen in mossy fiber sprouting in hippocampal slice cultures. Neuroscience 1999;91:1009-1016.Volkow N.D., Logan J., Fowler J.S. et al.: Association between age-related decline in brain dopamine activity and impairment in frontal and cingulate metabolism. Am J Psychiatry 2000;157:75-80.
West M.J.: Regionally specific loss of neurons in the aging human hippocampus. Neurobiol Aging 1993;14:287-293.
Woodruff-Pak D.S.: Eyeblink classical conditioning differentiates normal aging from Alzheimer's disease. Integr Physiol Behav Sci 2001;36:87-108.
Woodruff-Pak D.S., Cronholm J.F., Sheffield J.B.:
Purkinje cell number related to rate of classical conditioning. Neuroreport 1990;1:165-168.Woodruff-Pak D.S., Romano S., Papka M.: Training to criterion in eyeblink classical conditioning in Alzheimer's disease, Down's syndrome with Alzheimer's disease, and healthy elderly. Behav Neurosci 1996;110:22-29.
Woodruff-Pak D.S., Trojanowski J.Q.: The older rabbit as an animal model: implications for Alzheimer's disease. Neurobiol Aging 1996;17:283-290.
Woodruff-Pak D.S., Vogel R.Wr., Ewers M., Coffey J. et al.: Assessed volume of cerebellum correlates with associative learning. Neurobiol Learn Mem 2001;76:342-357.
Adv. Gerontol.-2002.-Vol. 10.-P. 35-39
Список литературы
1.Araque A., Carmignoto G., Haydon P.G.: Dynamic signaling between astrocytes and neurons. Ann. Rev. Physiol. 2001;63:795-813.
2.Arnsten A.F., Cai J.X., Steere J.C., Goldman-Rakic P.S.:
Dopamine D2 receptor mechanisms contribute to age-related cognitive decline: the effects of quinpirole on memory and motor performance in monkeys. J Neurosci 1995; 15:3429-3439.
3.Bi R., Foy M.R., Vouimba R.M. et al.: Cyclic changes in estradiol regulate synaptic plasticity through the MAP kinase pathway. Proc Natl Acad Sci U S A 2001;98:13391-13395.
4.Birren J.E. Englewood Cliffs, NJ, Prentice-Hall, 1964.
5.Carlson L.E., Sherwin B.B.: Higher levels of plasma estradiol and testosterone in healthy elderly men compared with age-matched women may protect aspects of explicit memory. Menopause 2000;7:168-177.
6.Costa S., Planchenault T., Charriere-Bertrand C. et al.:
Astroglial permissivityfor neuritic outgrowth in neuron-astrocyte cocultures depends on regulation of laminin bioavailability. Glia 2002;37:105-113.
7.Finch C.: Longevity, Senescence, and the Genome, U Chicago Press, 1990.
8.Finch C., Kirkwood T.B.L: Chance, Development, and Aging, Oxford University Press, 2000.
9.Finch C., Longo V.: The Gero-inflammatory manifold; in Roger J (ed): Neuroinflammatory Mechanisms in Alzheimer's Disease: Basic and Clinical Research, Basel: BirkhauserVerlag,
2000, pp Chapter 1.
10.Finch C., Morgan T.E., Rozovskyl., Xie Z. et al.: Microglia and aging in the brain.; in Streit WJ (ed): Microglia in the Regenerating and Degenerating Central Nervous System. New York, Springer-Verlag, 2002, pp 275-305.
11.Finch C.E., Pike M.C., Witten M.: Slow mortality rate accelerations during aging in some animals approximate that of humans. Science 1990;249:902-905.
12.Foy M.R., Xu J., Xie X. et al.: 17beta-estradiol enhances NMDA receptor-mediated EPSPs and long-term potentiation. J Neurophysiol 1999;81:925-929.
13.Garcia Segura L.M., Naftolin F., Hutchison J.B., et al.:
Role of astroglia in estrogen regulation of synaptic plasticity and brain repair. J Neurobiol 1999;40:574-584.
14.Gatz M., Pedersen N.L., Berg S. et al.: Heritability for Alzheimer's disease: the study of dementia in Swedish twins. J Gerontol A Biol Sci Med Sci 1997;52:M117-125.
15.GossJ.R., Finch C.E., Morgan D.G.: Age-related changes in glial fibrillary acidic protein mRNA in the mouse brain. Neurobiol Aging 1991;12:165-170.
16.Hall T.C., Miller A.K.H., Corsellis J.A.N.: Variations in the human Purkinje cell population according to age and sex. Neuropathol Appl Neurobiol 1975;1:267-292.
17.Hof P.R., Mobbs C.V.: Functional Neurobiology of Aging.
2001.
18.Hogervorst E., Williams J., Budge M., RiedelW., JollesJ.:
The nature of the effect of female gonadal hormone replacement therapy on cognitive function in post-menopausal women: a meta-analysis. Neuroscience 2000;101:485-512.
19.Inoue M., Suhara T., Sudo Y., Okubo Y. et al.: Age-related reduction of extrastriatal dopamine D2 receptor measured by PET. Life Sci 2001;69:1079-1084.
20.Keck B.J., Lakowski J.M.: Neurochemistry of receptor dynamics in the aging brain: in Hof PR, Mobbs CV (eds):
Functional Neurobiology of Aging. New York, Academic Press, 2001, pp 21-29.
21.Laping N.J., Teter B., Nichols N.R., Rozovsky I., Finch C.E.: Glial fibrillary acidic protein: regulation by hormones, cytokines, and growth factors. Brain Pathol 1994;4:259-275.
22.Lefrancois T., Fages C., Peschanski M., Tardy M.:
Neuritic outgrowth associated with astroglial phenotypic changes induced by antisense glial fibrillary acidic protein (GFAP) mRNA in injured neuron-astrocyte cocultures. J Neurosci 1997;17:4121-4128.
23.Lindenberger U., Marsiske M., Baltes P.B.: Memorizing while walking: increase in dual-task costs from young adulthood to old age. Psychol Aging 2000;15:417-436.
McClearn G.E., Johansson В., Berg S. et al.: Substantial genetic influence on cognitive abilities in twins 80 or more years old. Science 1997;276:1560-1563.
24.McMillian M.K., Thai L, Hong J.S. et al.: Brain injury in a dish: a model for reactive gliosis. Trends Neurosci 1994;17:138-142.
25.Meaney M.J., Aitken D.H., van Berkel C., Bhatnagar S., Sapolsky R.M.: Effect of neonatal handling on age-related impairments associated with the hippocampus. Science 1988;239:766-768.
26.Menet V., Gimenez у Ribotta M., Chauvet N. et al.:
Inactivation of the glial fibrillary acidic protein gene, but not that of vimentin, improves neuronal survival and neurite growth by modifying adhesion molecule expression. J Neurosci 2001;21:6147-6158.
27.Morgan D.G., Marcusson J.O., Nyberg P. et al.: Divergent changes in D-1 and D-2 dopamine binding sites in human brain during aging. Neurobiol Aging 1987;8:195-201.
28.Morgan Т.Е., Rozovsky I., Goldsmith S.K., et al.:
Increased transcription of the astrocyte gene GFAP during middle-age is attenuated by food restriction: implications for the role of oxidative stress. Free Radic Biol Med 1997;23:524-528.
29.Morgan Т.Е., Xie Z., Goldsmith S., Yoshida Т., et al.: The mosaic of brain glial hyperactivity during normal ageing and its attenuation by food restriction. Neuroscience 1999;89:687-699.
30.Nelson J.F., Felicio L.S.: Radical ovarian resection advances the onset of persistent vaginal cornification but only transiently disrupts hypothalamic-pituitary regulation of cyclicity in C57BL/6J mice. Biol Reprod 1986;35:957-964.
31.Nichols N.R., Day J.R., Laping N.J., Johnson S.A., Finch C.E.: GFAP mRNA increases with age in rat and human brain. Neurobiol Aging 1993;14:421-429.
32.Oliet S.H., Piet R., Poulain D.A.: Control of glutamate clearance and synaptic efficacy by glial coverage of neurons. Science 2001;292:923-926.
33.Plassman B.L, Welsh-Bohmer K.A., Bigler E.D. et al.:
Apolipoprotein E epsilon 4 allele and hippocampal volume in twins with normal cognition. Neurology 1997;48:985-989.
34.Rasmussen Т., Schliemann Т., Sorensen J.C. et al.:
Memory impaired aged rats: no loss of principal hippocampal and subicular neurons. Neurobiol Aging 1996;17:143-147.
35.Rogers J., Zornetzer S.F., Bloom F.E., Mervis R.E.:
Senescent microstructural changes in rat cerebellum. Brain Res 1984;292:23-32.
36.Rozovsky I., Wei M., Stone D.J., Zanjani H. et al.: Estradiol (E2) enhances neurite outgrowth by repressing glial fibrillary acidic protein expression and reorganizing laminin. Endocrinology 2002;143:636-646.
37.Salthouse Т.A.: Aging and measures of processing speed. Biol Psychol 2000;54:35-54.
38.Schneider L.S., Finch C.E.: Can estrogens prevent neurodegeneration? Drugs Aging 1997;11:87-95.
39.Severson J.A., Finch C.E.: Reduced dopaminergic binding during aging in the rodent striatum. Brain Res 1980;192:147-162.
40.Sohal R.S., Weindruch R.: Oxidative stress, caloric restriction, and aging. Science 1996;273:59-63.
41.Stein-Behrens В., Mattson M.P., Chang I., Yeh M., Sapolsky R.: Stress exacerbates neuron loss and cytoskeletal pathology in the hippocampus. J Neurosci 1994;14:5373-5380.
42.Stone D.J., Rozovsky I., Morgan Т.Е. et al.: Increased synaptic sprouting in response to estrogen via an apolipoprotein E-dependent mechanism: implications for Alzheimer's disease. J Neurosci 1998;18:3180-3185.
43.Stone D.J., Rozovsky I., Morgan Т.Е., Anderson C.P. et al.: Effects of age on gene expression during estrogen-induced synaptic sprouting in the female rat. Exp Neurol 2000;165:46-57.
44.Stone D.J., Song Y., Anderson C.P., Krohn K.K., Finch C.E., Rozovsky I.: Bidirectional transcription regulation of glial fibrillary acidic protein by estradiol in vivo and in vitro. Endocrinology 1998; 139:3202-3209.
45.Suzuki M., Hatano К., Sakiyama Y. et al.: Age-related changes of dopamine D1-like and D2-like receptor binding in the F344/N rat striatum revealed by positron emission tomography and in vitro receptor autoradiography. Synapse 2001;41:285-293.
46.Teter В., Harris-White M.E., Frautschy S.A., Cole G.M.:
Role of apolipoprotein E and estrogen in mossy fiber sprouting in hippocampal slice cultures. Neuroscience 1999;91:1009-1016.
47.Volkow N.D., Logan J., Fowler J.S. et al.: Association between age-related decline in brain dopamine activity and impairment in frontal and cingulate metabolism. Am J Psychiatry 2000;157:75-80.
48.West M.J.: Regionally specific loss of neurons in the aging human hippocampus. Neurobiol Aging 1993;14:287-293.
49.Woodruff-Pak D.S.: Eyeblink classical conditioning differentiates normal aging from Alzheimer's disease. Integr Physiol Behav Sci 2001;36:87-108.
50.Woodruff-Pak D.S., Cronholm J.F., Sheffield J.B.:
Purkinje cell number related to rate of classical conditioning. Neuroreport 1990;1:165-168.
51.Woodruff-Pak D.S., Romano S., Papka M.: Training to criterion in eyeblink classical conditioning in Alzheimer's disease, Down's syndrome with Alzheimer's disease, and healthy elderly. Behav Neurosci 1996;110:22-29.
52.Woodruff-Pak D.S., Trojanowski J.Q.: The older rabbit as an animal model: implications for Alzheimer's disease. Neurobiol Aging 1996;17:283-290.
53.Woodruff-Pak D.S., Vogel R.Wr., Ewers M., Coffey J. et al.: Assessed volume of cerebellum correlates with associative learning. Neurobiol Learn Mem 2001;76:342-357.
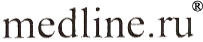